It’s interesting to imagine
ourselves with abilities that far surpass what a human can do. Just
visualize yourself lifting 10 times your own weight or being able to see
radiation with your own eyes.
In a way, that’s not as
crazy as it might sound. If it were possible to implant certain animal
organs into our own bodies, they could give us these powers and much
more.
10 Planarian Cells
First, let us look at the planarian’s cells. These...
Wednesday, November 21, 2018
Sunday, June 24, 2018
Posted by Sagar on 2:34 AM with No comments
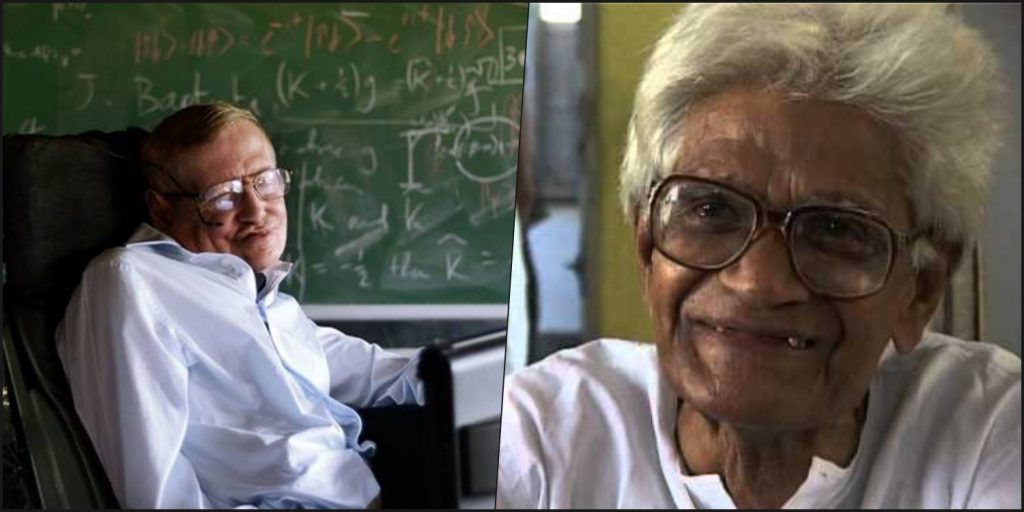
The Little Known Calcutta Scientist Whose Shoulders Hawking Stood On
Amal Kumar Raychaudhuri described the dynamics of light’s motion through the curved parts of spacetime. It was “perhaps the single most important input” for one of Hawking’s major findings.
Stephen Hawking (left; source: hawking.org.uk) and Amal Kumar Raychaudhuri (source: YouTube)
One of Stephen Hawking’s more celebrated contributions to physics had to do with...
Saturday, March 17, 2018
Sunday, February 18, 2018
Wednesday, January 31, 2018
Friday, January 12, 2018
Posted by Sagar on 9:41 AM with No comments

The 11 Greatest Unanswered Questions of Physics
http://royalphysicist.blogspot.com/2018/01/the-11-greatest-unanswered-questions-of.html
By Sagar Rawal
By Sagar Rawal
Here's a tale of modern physics: Two scientists work at the same university in different fields. One studies huge objects far from Earth. The other is fascinated by the tiny stuff right in front of him. To satisfy their curiosities, one builds the world's most...
Posted by Sagar on 9:31 AM with No comments

8 Ways to Win the Nobel Prize in Physics
http://royalphysicist.blogspot.com/2018/01/8-ways-to-win-nobel-prize-in-physics.html
By Sagar Rawal
It’s 5 a.m. and you’re sitting by the phone, hoping for that “magic call” from Stockholm that bears the news you’ve waited so long for: You’ve won the Nobel Prize in Physics! Whom will you tell first? How will you celebrate? And what color Bugatti should you buy with your prize money?1 But...
Tuesday, January 9, 2018
Posted by Sagar on 10:24 AM with No comments

Why an Old Theory of Everything Is Gaining New Life
Twenty-five particles and four forces. That description — the Standard Model of particle physics — constitutes physicists’ best current explanation for everything. It’s neat and it’s simple, but no one is entirely happy with it. What irritates physicists most is that one of the forces — gravity — sticks out like a sore thumb on a four-fingered hand. Gravity is different.
Unlike...
Subscribe to:
Posts (Atom)